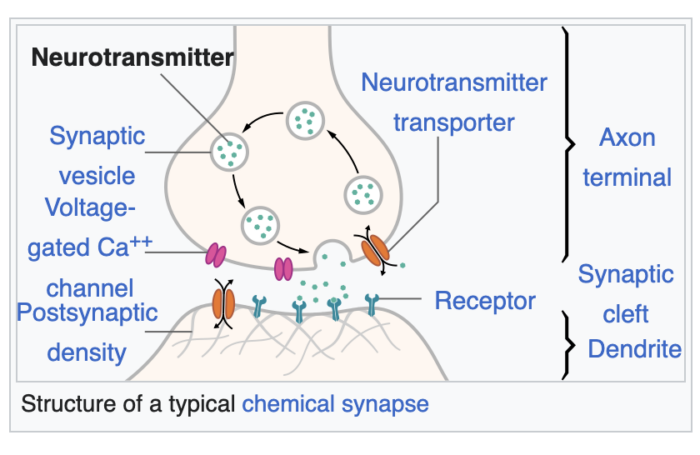
.
.
*based on their ‘structural/functional characteristics’, the ‘neuro-transmitter receptor’ can be classified into 2 broad categories…*
#1
*METABO-TROPIC RECEPTORS*
A metabotropic receptor is a type of membrane receptor of eukaryotic cells that acts through a 2nd messenger
It may be located at the surface of the cell or in vesicles
.
#2
*IONO-TROPIC RECEPTORS*
ionotropic receptors form an ion channel pore
.
In contrast, metabotropic receptors are indirectly linked with ion channels on the plasma membrane of the cell through signal transduction mechanisms, often G proteins.
Hence, G protein-coupled receptors are inherently metabotropic.
Other examples of metabotropic receptors include tyrosine kinases and guanylyl cyclase receptors
Both receptor types are activated by specific neurotransmitters.
When an ionotropic receptor is activated, it opens a channel that allows ions such as Na+, K+, or Cl− to flow.
In contrast, when a metabotropic receptor is activated, a series of intracellular events are triggered that can also result in ion channels opening or other intracellular events, but involve a range of second messenger chemicals.[2]
Contents
Structure
The G protein-coupled receptors have seven hydrophobic transmembrane domains.
Most of them are monomeric proteins, although GABAB receptors require heterodimerization to function properly. The protein’s N terminus is located on the extracellular side of the membrane and its C terminus is on the intracellular side.[2]
The 7 transmembrane spanning domains, with an external amino terminus, are often claimed as being alpha helix shaped, and the polypeptide chain is said to be composed of ~ 450-550 amino acids
.
Function
Metabotropic receptors have neurotransmitters as ligands, which, when bound to the receptors, initiate cascades that can lead to channel-opening or other cellular effects.
When a ligand (the neurotransmitter) binds to the receptor (the transducer) the latter activates a primary effector via the G-protein, which can go on to activate secondary messengers or have other effects. Since opening channels by metabotropic receptors involves activating a number of molecules in turn, channels associated with these receptors take longer to open than ionotropic receptors do, and they are thus not involved in mechanisms that require quick responses.[3]:240 However, metabotropic receptors also remain open from seconds to minutes.[3]:250–1 Thus they have a much longer-lasting effect than ionotropic receptors, which open quickly but only remain open for a few milliseconds.[1] While ionotropic channels have an effect only in the immediate region of the receptor, the effects of metabotropic receptors can be more widespread through the cell.
Metabotropic receptors can either open or close channels in the cell membrane. They can make a membrane more excitable by closing K+ channels, retaining positive charge within the cell and thus reducing the amount of current necessary to cause an action potential.[3]:242–3 Metabotropic receptors on the presynaptic membrane can inhibit or, more rarely, facilitate neurotransmitter release from the presynaptic neuron.[4]
These receptors can be further classified into receptor tyrosine kinases and G protein-coupled receptors, or GPCRs.[3]:229
.
.
*LIGAND-GATED ION CHANNELS*
Ligand-gated ion channel – Wikipedia
LGIC.png
When ligands bind to the receptor, the ion channel portion of the receptor opens, allowing ions to pass across the cell membrane.
Ligand-gated ion channels (LICs, LGIC), also commonly referred to as ionotropic receptors, are a group of transmembrane ion-channel proteins which open to allow ions such as Na+, K+, Ca2+, and/or Cl− to pass through the membrane in response to the binding of a chemical messenger (i.e. a ligand), such as a neurotransmitter.[1][2][3]
When a presynaptic neuron is excited, it releases a neurotransmitter from vesicles into the synaptic cleft. The neurotransmitter then binds to receptors located on the postsynaptic neuron. If these receptors are ligand-gated ion channels, a resulting conformational change opens the ion channels, which leads to a flow of ions across the cell membrane. This, in turn, results in either a depolarization, for an excitatory receptor response, or a hyperpolarization, for an inhibitory response.
These receptor proteins are typically composed of at least two different domains: a transmembrane domain which includes the ion pore, and an extracellular domain which includes the ligand binding location (an allosteric binding site). This modularity has enabled a ‘divide and conquer’ approach to finding the structure of the proteins (crystallising each domain separately). The function of such receptors located at synapses is to convert the chemical signal of presynaptically released neurotransmitter directly and very quickly into a postsynaptic electrical signal. Many LICs are additionally modulated by allosteric ligands, by channel blockers, ions, or the membrane potential. LICs are classified into three superfamilies which lack evolutionary relationship: cys-loop receptors, ionotropic glutamate receptors and ATP-gated channels.
Contents
Cys-loop receptors
Nicotinic acetylcholine receptor in closed state with predicted membrane boundaries shown, PDB 2BG9
The cys-loop receptors are named after a characteristic loop formed by a disulfide bond between two cysteine residues in the N terminal extracellular domain. They are part of a larger family of pentameric ligand-gated ion channels that usually lack this disulfide bond, hence the tentative name “Pro-loop receptors”.[4][5] A binding site in the extracellular N-terminal ligand-binding domain gives them receptor specificity for (1) acetylcholine (AcCh), (2) serotonin, (3) glycine, (4) glutamate and (5) γ-aminobutyric acid (GABA) in vertebrates. The receptors are subdivided with respect to the type of ion that they conduct (anionic or cationic) and further into families defined by the endogenous ligand. They are usually pentameric with each subunit containing 4 transmembrane helices constituting the transmembrane domain, and a beta sheet sandwich type, extracellular, N terminal, ligand binding domain.[6] Some also contain an intracellular domain like shown in the image.
The prototypic ligand-gated ion channel is the nicotinic acetylcholine receptor. It consists of a pentamer of protein subunits (typically ααβγδ), with two binding sites for acetylcholine (one at the interface of each alpha subunit). When the acetylcholine binds it alters the receptor’s configuration (twists the T2 helices which moves the leucine residues, which block the pore, out of the channel pathway) and causes the constriction in the pore of approximately 3 angstroms to widen to approximately 8 angstroms so that ions can pass through. This pore allows Na+ ions to flow down their electrochemical gradient into the cell. With a sufficient number of channels opening at once, the inward flow of positive charges carried by Na+ ions depolarizes the postsynaptic membrane sufficiently to initiate an action potential.
While single-cell organisms like bacteria would have little apparent need for the transmission of an action potential, a bacterial homologue to an LIC has been identified, hypothesized to act nonetheless as a chemoreceptor.[4] This prokaryotic nAChR variant is known as the GLIC receptor, after the species in which it was identified; Gloeobacter Ligand-gated Ion C channel.
Structure
Cys-loop receptors have structural elements that are well conserved, with a large extracellular domain (ECD) harboring an alpha-helix and 10 beta-strands. Following the ECD, four transmembrane segments (TMSs) are connected by intracellular and extracellular loop structures.[7] Except the TMS 3-4 loop, their lengths are only 7-14 residues. The TMS 3-4 loop forms the largest part of the intracellular domain (ICD) and exhibits the most variable region between all of these homologous receptors. The ICD is defined by the TMS 3-4 loop together with the TMS 1-2 loop preceding the ion channel pore.[7] Crystallization has revealed structures for some members of the family, but to allow crystallization, the intracellular loop was usually replaced by a short linker present in prokaryotic cys-loop receptors, so their structures as not known. Nevertheless, this intracellular loop appears to function in desensitization, modulation of channel physiology by pharmacological substances, and posttranslational modifications. Motifs important for trafficking are therein, and the ICD interacts with scaffold proteins enabling inhibitory synapse formation.[7]
Cationic cys-loop receptors
Type Class IUPHAR-recommended
protein name [8] Gene Previous names
Serotonin
(5-HT) 5-HT3 5-HT3A
5-HT3B
5-HT3C
5-HT3D
5-HT3E HTR3A
HTR3B
HTR3C
HTR3D
HTR3E 5-HT3A
5-HT3B
5-HT3C
5-HT3D
5-HT3E
Nicotinic acetylcholine
(nAChR) alpha α1
α2
α3
α4
α5
α6
α7
α9
α10 CHRNA1
CHRNA2
CHRNA3
CHRNA4
CHRNA5
CHRNA6
CHRNA7
CHRNA9
CHRNA10 ACHRA, ACHRD, CHRNA, CMS2A, FCCMS, SCCMS
beta β1
β2
β3
β4 CHRNB1
CHRNB2
CHRNB3
CHRNB4 CMS2A, SCCMS, ACHRB, CHRNB, CMS1D
EFNL3, nAChRB2
gamma γ CHRNG ACHRG
delta δ CHRND ACHRD, CMS2A, FCCMS, SCCMS
epsilon ε CHRNE ACHRE, CMS1D, CMS1E, CMS2A, FCCMS, SCCMS
Zinc-activated ion channel
(ZAC) ZAC ZACN ZAC1, L2m LICZ, LICZ1
Anionic cys-loop receptors
Type Class IUPHAR-recommended
protein name[8] Gene Previous names
GABAA alpha α1
α2
α3
α4
α5
α6 GABRA1
GABRA2
GABRA3
GABRA4
GABRA5
GABRA6 EJM, ECA4
beta β1
β2
β3 GABRB1
GABRB2
GABRB3
ECA5
gamma γ1
γ2
γ3
GABRG1
GABRG2
GABRG3 CAE2, ECA2, GEFSP3
delta δ GABRD
epsilon ε GABRE
pi π GABRP
theta θ GABRQ
rho ρ1
ρ2
ρ3 GABRR1
GABRR2
GABRR3 GABAC[9]
Glycine
(GlyR) alpha α1
α2
α3
α4 GLRA1
GLRA2
GLRA3
GLRA4 STHE
beta β GLRB
Ionotropic glutamate receptors
The ionotropic glutamate receptors bind the neurotransmitter glutamate. They form tetramers with each subunit consisting of an extracellular amino terminal domain (ATD, which is involved tetramer assembly), an extracellular ligand binding domain (LBD, which binds glutamate), and a transmembrane domain (TMD, which forms the ion channel). The transmembrane domain of each subunit contains three transmembrane helices as well as a half membrane helix with a reentrant loop. The structure of the protein starts with the ATD at the N terminus followed by the first half of the LBD which is interrupted by helices 1,2 and 3 of the TMD before continuing with the final half of the LBD and then finishing with helix 4 of the TMD at the C terminus. This means there are three links between the TMD and the extracellular domains. Each subunit of the tetramer has a binding site for glutamate formed by the two LBD sections forming a clamshell like shape. Only two of these sites in the tetramer need to be occupied to open the ion channel. The pore is mainly formed by the half helix 2 in a way which resembles an inverted potassium channel.
Type Class IUPHAR-recommended
protein name [8] Gene Previous names
AMPA GluA GluA1
GluA2
GluA3
GluA4 GRIA1
GRIA2
GRIA3
GRIA4 GLUA1, GluR1, GluRA, GluR-A, GluR-K1, HBGR1
GLUA2, GluR2, GluRB, GluR-B, GluR-K2, HBGR2
GLUA3, GluR3, GluRC, GluR-C, GluR-K3
GLUA4, GluR4, GluRD, GluR-D
Kainate GluK GluK1
GluK2
GluK3
GluK4
GluK5 GRIK1
GRIK2
GRIK3
GRIK4
GRIK5 GLUK5, GluR5, GluR-5, EAA3
GLUK6, GluR6, GluR-6, EAA4
GLUK7, GluR7, GluR-7, EAA5
GLUK1, KA1, KA-1, EAA1
GLUK2, KA2, KA-2, EAA2
NMDA GluN GluN1
NRL1A
NRL1B GRIN1
GRINL1A
GRINL1B GLUN1, NMDA-R1, NR1, GluRξ1
GluN2A
GluN2B
GluN2C
GluN2D GRIN2A
GRIN2B
GRIN2C
GRIN2D GLUN2A, NMDA-R2A, NR2A, GluRε1
GLUN2B, NMDA-R2B, NR2B, hNR3, GluRε2
GLUN2C, NMDA-R2C, NR2C, GluRε3
GLUN2D, NMDA-R2D, NR2D, GluRε4
GluN3A
GluN3B GRIN3A
GRIN3B GLUN3A, NMDA-R3A, NMDAR-L, chi-1
GLU3B, NMDA-R3B
‘Orphan’ (GluD) GluD1
GluD2 GRID1
GRID2 GluRδ1
GluRδ2
AMPA receptor
The AMPA receptor bound to a glutamate antagonist showing the amino terminal, ligand binding, and transmembrane domain, PDB 3KG2
The α-amino-3-hydroxy-5-methyl-4-isoxazolepropionic acid receptor (also known as AMPA receptor, or quisqualate receptor) is a non-NMDA-type ionotropic transmembrane receptor for glutamate that mediates fast synaptic transmission in the central nervous system (CNS). Its name is derived from its ability to be activated by the artificial glutamate analog AMPA. The receptor was first named the “quisqualate receptor” by Watkins and colleagues after a naturally occurring agonist quisqualate and was only later given the label “AMPA receptor” after the selective agonist developed by Tage Honore and colleagues at the Royal Danish School of Pharmacy in Copenhagen.[10] AMPARs are found in many parts of the brain and are the most commonly found receptor in the nervous system. The AMPA receptor GluA2 (GluR2) tetramer was the first glutamate receptor ion channel to be crystallized.
AMPA receptor trafficking
Ligands:
NMDA receptors
Stylized depiction of an activated NMDAR
The N-methyl-D-aspartate receptor (NMDA receptor) – a type of ionotropic glutamate receptor – is a ligand-gated ion channel that is gated by the simultaneous binding of glutamate and a co-agonist (i.e., either D-serine or glycine).[11] Studies show that the NMDA receptor is involved in regulating synaptic plasticity and memory.[12][13]
The name “NMDA receptor” is derived from the ligand N-methyl-D-aspartate (NMDA), which acts as a selective agonist at these receptors. When the NMDA receptor is activated by the binding of two co-agonists, the cation channel opens, allowing Na+ and Ca2+ to flow into the cell, in turn raising the cell’s electric potential. Thus, the NMDA receptor is an excitatory receptor. At resting potentials, the binding of Mg2+ or Zn2+ at their extracellular binding sites on the receptor blocks ion flux through the NMDA receptor channel. “However, when neurons are depolarized, for example, by intense activation of colocalized postsynaptic AMPA receptors, the voltage-dependent block by Mg2+ is partially relieved, allowing ion influx through activated NMDA receptors. The resulting Ca2+ influx can trigger a variety of intracellular signaling cascades, which can ultimately change neuronal function through activation of various kinases and phosphatases”.[14]
Ligands:
GABA receptors
GABA receptors are major inhibitory neurotransmitter expressed in the major interneurons in animal cortex.
GABAA receptor
GABAA receptor schematic
GABAA receptors are ligand-gated ion channels. GABA (gamma-aminobutyric acid), the endogenous ligand for these receptors, is the major inhibitory neurotransmitter in the central nervous system. When activated, it mediates Cl– flow into the neuron, hyperpolarizing the neuron. GABAA receptors occur in all organisms that have a nervous system. Due to their wide distribution within the nervous system of mammals, they play a role in virtually all brain functions.[16]
Various ligands can bind specifically to GABAA receptors, either activating or inhibiting the Cl– channel.
Ligands:
5-HT3 receptor
5-HT3 receptor
The pentameric 5-HT3 receptor is permeable to sodium (Na), potassium (K), and calcium (Ca) ions.
ATP-gated channels
Figure 1. Schematic representation showing the membrane topology of a typical P2X receptor subunit. First and second transmembrane domains are labeled TM1 and TM2.
Main article: P2X receptor
ATP-gated channels open in response to binding the nucleotide ATP. They form trimers with two transmembrane helices per subunit and both the C and N termini on the intracellular side.
Type Class IUPHAR-recommended
protein name [8] Gene Previous names
P2X N/A P2X1
P2X2
P2X3
P2X4
P2X5
P2X6
P2X7 P2RX1
P2RX2
P2RX3
P2RX4
P2RX5
P2RX6
P2RX7 P2X1
P2X2
P2X3
P2X4
P2X5
P2X6
P2X7
PIP2-gated channels
Phosphatidylinositol 4,5-bisphosphate (PIP2) binds to and directly activates inwardly rectifying potassium channels (Kir).[17] PIP2 is a cell membrane lipid, and its role in gating ion channels represents a novel role for the molecule.[18][19]
Indirect modulation
In contrast to ligand-gated ion channels, there are also receptor systems in which the receptor and the ion channel are separate proteins in the cell membrane, instead of a single molecule. In this case, ion channels are indirectly modulated by activation of the receptor, instead of being gated directly.
G-protein-linked receptors
G-protein-coupled receptor mechanism
Also called G protein-coupled receptor, seven-transmembrane domain receptor, 7 TM receptor, constitute a large protein family of receptors that sense molecules outside the cell and activate inside signal transduction pathways and, ultimately, cellular responses. They pass through the cell membrane 7 times. G-protein-Linked receptors are a huge family that have hundreds of members identified. Ion-channel-linked receptors (e.g. GABAB, NMDA, etc.) are only a part of them.
Table 1. Three major families of Trimeric G Proteins[20]
FAMILY SOME FAMILY MEMBERS ACTION MEDIATED BY FUNCTIONS
I GS α Activate adenylyl cyclase activates Ca2+ channels
Golf α Activates adenylyl cyclase in olfactory sensory neurons
II Gi α Inhibits adenylyl cyclase
βɣ Activates K+ channels
G0 βɣ Activates K+ channels; inactivate Ca2+ channels
α and βɣ Activates phospholipase C-β
Gt (transducin) α Activate cyclic GMP phosphodiesterase in vertebrate rod photoreceptors
III Gq α Activates phospholipase C-β
GABAB receptor
GABAB receptors are metabotropic transmembrane receptors for gamma-aminobutyric acid. They are linked via G-proteins to K+ channels, when active, they create hyperpolarized effect and lower the potential inside the cell.[21]
Ligands:
Gα signaling
The cyclic-adenosine monophosphate (cAMP)-generating enzyme adenylate cyclase is the effector of both the Gαs and Gαi/o pathways. Ten different AC gene products in mammals, each with subtle differences in tissue distribution and/or function, all catalyze the conversion of cytosolic adenosine triphosphate (ATP) to cAMP, and all are directly stimulated by G-proteins of the Gαs class. Interaction with Gα subunits of the Gαi/o type, on the contrary, inhibits AC from generating cAMP. Thus, a GPCR coupled to Gαs counteracts the actions of a GPCR coupled to Gαi/o, and vice versa. The level of cytosolic cAMP may then determine the activity of various ion channels as well as members of the ser/thr-specific protein kinase A (PKA) family. As a result, cAMP is considered a second messenger and PKA a secondary effector.
The effector of the Gαq/11 pathway is phospholipase C-β (PLCβ), which catalyzes the cleavage of membrane-bound phosphatidylinositol 4,5-biphosphate (PIP2) into the second messengers inositol (1,4,5) trisphosphate (IP3) and diacylglycerol (DAG). IP3 acts on IP3 receptors found in the membrane of the endoplasmic reticulum (ER) to elicit Ca2+ release from the ER, DAG diffuses along the plasma membrane where it may activate any membrane localized forms of a second ser/thr kinase called protein kinase C (PKC). Since many isoforms of PKC are also activated by increases in intracellular Ca2+, both these pathways can also converge on each other to signal through the same secondary effector. Elevated intracellular Ca2+ also binds and allosterically activates proteins called calmodulins, which in turn go on to bind and allosterically activate enzymes such as Ca2+/calmodulin-dependent kinases (CAMKs).
The effectors of the Gα12/13 pathway are three RhoGEFs (p115-RhoGEF, PDZ-RhoGEF, and LARG), which, when bound to Gα12/13 allosterically activate the cytosolic small GTPase, Rho. Once bound to GTP, Rho can then go on to activate various proteins responsible for cytoskeleton regulation such as Rho-kinase (ROCK). Most GPCRs that couple to Gα12/13 also couple to other sub-classes, often Gαq/11.
Gβγ signaling
The above descriptions ignore the effects of Gβγ–signalling, which can also be important, in particular in the case of activated Gαi/o-coupled GPCRs. The primary effectors of Gβγ are various ion channels, such as G-protein-regulated inwardly rectifying K+ channels (GIRKs), P/Q- and N-type voltage-gated Ca2+ channels, as well as some isoforms of AC and PLC, along with some phosphoinositide-3-kinase (PI3K) isoforms.
Clinical relevance
Ligand-gated ion channels are likely to be the major site at which anaesthetic agents and ethanol have their effects, although unequivocal evidence of this is yet to be established.[23][24] In particular, the GABA and NMDA receptors are affected by anaesthetic agents at concentrations similar to those used in clinical anaesthesia.[25]
By understanding the mechanism and exploring the chemical/biological/physical component that could function on those receptors, more and more clinical applications are proven by preliminary experiments or FDA.
.
*AKA* —>
“chemical messengers”
.
(“neurotransmitters” are ‘endogenous’ chemicals that enable ‘neurotransmission’)
(they transmit signals across a chemical synapse, such as a neuromuscular junction, from one neuron (nerve cell) to another “target” neuron, muscle cell, or gland cell)
(neurotransmitters are released from synaptic vesicles in synapses into the synaptic cleft, where they are received by receptors on the target cells)
(many neurotransmitters are synthesized from simple and plentiful precursors such as amino acids, which are readily available from the diet and only require a small number of biosynthetic steps for conversion)
(neurotransmitters play a major role in shaping everyday life and functions)
.
(their exact numbers are unknown, but more than ‘100 chemical messengers’ have been uniquely identified)
.
Neurotransmitter – Wikipedia
An illustrated chemical synapse
Neurotransmitters are endogenous chemicals that enable neurotransmission. It is a type of chemical messenger which transmits signals across a chemical synapse, such as a neuromuscular junction, from one neuron (nerve cell) to another “target” neuron, muscle cell, or gland cell.[1] Neurotransmitters are released from synaptic vesicles in synapses into the synaptic cleft, where they are received by neurotransmitter receptors on the target cells. Many neurotransmitters are synthesized from simple and plentiful precursors such as amino acids, which are readily available from the diet and only require a small number of biosynthetic steps for conversion. Neurotransmitters play a major role in shaping everyday life and functions. Their exact numbers are unknown, but more than 200 unique chemical messengers have been identified.[2][3][4]
Contents
Mechanism
Synaptic vesicles containing neurotransmitters
Neurotransmitters are stored in synaptic vesicles, clustered close to the cell membrane at the axon terminal of the presynaptic neuron. Neurotransmitters are released into and diffuse across the synaptic cleft, where they bind to specific receptors on the membrane of the postsynaptic neuron.[5]
Most neurotransmitters are about the size of a single amino acid; however, some neurotransmitters may be the size of larger proteins or peptides. A released neurotransmitter is typically available in the synaptic cleft for a short time before it is metabolized by enzymes, pulled back into the presynaptic neuron through reuptake, or bound to a postsynaptic receptor. Nevertheless, short-term exposure of the receptor to a neurotransmitter is typically sufficient for causing a postsynaptic response by way of synaptic transmission.
In response to a threshold action potential or graded electrical potential, a neurotransmitter is released at the presynaptic terminal. Low level “baseline” release also occurs without electrical stimulation. The released neurotransmitter may then move across the synapse to be detected by and bind with receptors in the postsynaptic neuron. Binding of neurotransmitters may influence the postsynaptic neuron in either an inhibitory or excitatory way. This neuron may be connected to many more neurons, and if the total of excitatory influences are greater than those of inhibitory influences, the neuron will also “fire”. Ultimately it will create a new action potential at its axon hillock to release neurotransmitters and pass on the information to yet another neighbouring neuron.[6]
Until the early 20th century, scientists assumed that the majority of synaptic communication in the brain was electrical. However, through the careful histological examinations by Ramón y Cajal (1852–1934), a 20 to 40 nm gap between neurons, known today as the synaptic cleft, was discovered. The presence of such a gap suggested communication via chemical messengers traversing the synaptic cleft, and in 1921 German pharmacologist Otto Loewi (1873–1961) confirmed that neurons can communicate by releasing chemicals. Through a series of experiments involving the vagus nerves of frogs, Loewi was able to manually slow the heart rate of frogs by controlling the amount of saline solution present around the vagus nerve. Upon completion of this experiment, Loewi asserted that sympathetic regulation of cardiac function can be mediated through changes in chemical concentrations. Furthermore, Otto Loewi is credited with discovering acetylcholine (ACh)—the first known neurotransmitter.[7] Some neurons do, however, communicate via electrical synapses through the use of gap junctions, which allow specific ions to pass directly from one cell to another.[8]
Identification
There are four main criteria for identifying neurotransmitters:
The chemical must be synthesized in the neuron or otherwise be present in it.
When the neuron is active, the chemical must be released and produce a response in some targets.
The same response must be obtained when the chemical is experimentally placed on the target.
A mechanism must exist for removing the chemical from its site of activation after its work is done.
However, given advances in pharmacology, genetics, and chemical neuroanatomy, the term “neurotransmitter” can be applied to chemicals that:
Carry messages between neurons via influence on the postsynaptic membrane.
Have little or no effect on membrane voltage, but have a common carrying function such as changing the structure of the synapse.
Communicate by sending reverse-direction messages that affect the release or reuptake of transmitters.
The anatomical localization of neurotransmitters is typically determined using immunocytochemical techniques, which identify the location of either the transmitter substances themselves, or of the enzymes that are involved in their synthesis. Immunocytochemical techniques have also revealed that many transmitters, particularly the neuropeptides, are co-localized, that is, one neuron may release more than one transmitter from its synaptic terminal.[9] Various techniques and experiments such as staining, stimulating, and collecting can be used to identify neurotransmitters throughout the central nervous system.[10]
Types
There are many different ways to classify neurotransmitters. Dividing them into amino acids, peptides, and monoamines is sufficient for some classification purposes.
Major neurotransmitters:
In addition, over 50 neuroactive peptides have been found, and new ones are discovered regularly. Many of these are “co-released” along with a small-molecule transmitter. Nevertheless, in some cases a peptide is the primary transmitter at a synapse. β-endorphin is a relatively well-known example of a peptide neurotransmitter because it engages in highly specific interactions with opioid receptors in the central nervous system.
Single ions (such as synaptically released zinc) are also considered neurotransmitters by some,[12] as well as some gaseous molecules such as nitric oxide (NO), carbon monoxide (CO), and hydrogen sulfide (H2S).[13] The gases are produced in the neural cytoplasm and are immediately diffused through the cell membrane into the extracellular fluid and into nearby cells to stimulate production of second messengers. Soluble gas neurotransmitters are difficult to study, as they act rapidly and are immediately broken down, existing for only a few seconds.
The most prevalent transmitter is glutamate, which is excitatory at well over 90% of the synapses in the human brain.[6] The next most prevalent is Gamma-Aminobutyric Acid, or GABA, which is inhibitory at more than 90% of the synapses that do not use glutamate. Although other transmitters are used in fewer synapses, they may be very important functionally: the great majority of psychoactive drugs exert their effects by altering the actions of some neurotransmitter systems, often acting through transmitters other than glutamate or GABA. Addictive drugs such as cocaine and amphetamines exert their effects primarily on the dopamine system. The addictive opiate drugs exert their effects primarily as functional analogs of opioid peptides, which, in turn, regulate dopamine levels.
List of neurotransmitters, peptides, and gaseous signaling molecules
This list is incomplete; you can help by expanding it.
Neurotransmitters
Category Name Abbreviation Metabotropic Ionotropic
Small: Amino acids (Arg) Arginine α2-Adrenergic receptors, imidazoline receptors NMDA receptors
Small: Amino acids Aspartate Asp – NMDA receptors
Small: Amino acids Glutamate Glu Metabotropic glutamate receptors NMDA receptors, kainate receptors, AMPARs
Small: Amino acids Gamma-aminobutyric acid GABA GABAB receptors GABAA receptors, GABAA-ρ receptors
Small: Amino acids Glycine Gly – NMDA receptors, glycine receptors
Small: Amino acids D-serine Ser – NMDA receptors
Small: Acetylcholine Acetylcholine Ach Muscarinic acetylcholine receptors Nicotinic acetylcholine receptors
Small: Monoamine (Phe/Tyr) Dopamine DA Dopamine receptors, trace amine-associated receptor 1[14][15] –
Small: Monoamine (Phe/Tyr) Norepinephrine (noradrenaline) NE, NAd Adrenergic receptors –
Small: Monoamine (Phe/Tyr) Epinephrine (adrenaline) Epi, Ad Adrenergic receptors –
Small: Monoamine (Trp) Serotonin (5-hydroxytryptamine) 5-HT Serotonin receptors (all except 5-HT3) 5-HT3
Small: Monoamine (His) Histamine H Histamine receptors –
Small: Trace amine (Phe) Phenethylamine PEA Human trace amine-associated receptors: hTAAR1, hTAAR2 –
Small: Trace amine (Phe) N-methylphenethylamine NMPEA hTAAR1 –
Small: Trace amine (Phe/Tyr) Tyramine TYR hTAAR1, hTAAR2 –
Small: Trace amine (Phe/Tyr) octopamine Oct hTAAR1 –
Small: Trace amine (Phe/Tyr) Synephrine Syn hTAAR1 –
Small: Trace amine (Trp) Tryptamine hTAAR1, various serotonin receptors –
Small: Trace amine (Trp) N-methyltryptamine NMT hTAAR1, various serotonin receptors –
Lipid Anandamide AEA Cannabinoid receptors –
Lipid 2-Arachidonoylglycerol 2-AG Cannabinoid receptors –
Lipid 2-Arachidonyl glyceryl ether 2-AGE Cannabinoid receptors –
Lipid N-Arachidonoyl dopamine NADA Cannabinoid receptors TRPV1
Lipid Virodhamine Cannabinoid receptors –
Small: Purine Adenosine Ado Adenosine receptors –
Small: Purine Adenosine triphosphate ATP P2Y receptors P2X receptors
Small: Purine Nicotinamide adenine dinucleotide β-NAD P2Y receptors P2X receptors
Neuropeptides
Category Name Abbreviation Metabotropic Ionotropic
Bombesin-like peptides Bombesin BBR1-2-3 –
Bombesin-like peptide Gastrin releasing peptide GRP – –
Bombesin-like peptide Neuromedin B NMB Neuromedin B receptor –
Bradykinins Bradykinin B1, B2 –
Calcitonin/CGRP family Calcitonin Calcitonin receptor –
Calcitonin/CGRP family Calcitonin gene-related peptide CGRP CALCRL –
Corticotropin-releasing factors Corticotropin-releasing hormone CRH CRHR1 –
Corticotropin-releasing factors Urocortin CRHR1 –
Galanins Galanin GALR1, GALR2, GALR3 –
Galanins Galanin-like peptide GALR1, GALR2, GALR3 –
Gastrins Gastrin Cholecystokinin B receptor –
Gastrins Cholecystokinin CCK Cholecystokinin receptors –
Melanocortins Adrenocorticotropic hormone ACTH ACTH receptor –
Melanocortins Proopiomelanocortin POMC Melanocortin 4 receptor –
Melanocortins Melanocyte-stimulating hormones MSH Melanocortin receptors –
Neurohypophyseals Vasopressin AVP Vasopressin receptors –
Neurohypophyseals Oxytocin OT Oxytocin receptor –
Neurohypophyseals Neurophysin I – –
Neurohypophyseals Neurophysin II – –
Neuromedins Neuromedin U NmU NmUR1, NmUR2 –
Neuropeptide B/W Neuropeptide B NPB NPBW1, NPBW2 –
Neuropeptide B/W Neuropeptide S NPS Neuropeptide S receptors –
Neuropeptide Y Neuropeptide Y NY Neuropeptide Y receptors –
Neuropeptide Y Pancreatic polypeptide PP – –
Neuropeptide Y Peptide YY PYY – –
Opioids Enkephalins δ-Opioid receptor –
Opioids Dynorphins κ-Opioid receptor –
Opioids Neoendorphins κ-Opioid receptor –
Opioids Endorphins μ-Opioid receptors –
Opioids Endomorphins μ-Opioid receptors –
Opioids Morphine μ-Opioid receptors –
Opioids Nociceptin/orphanin FQ N/OFQ Nociceptin receptors –
Orexins Orexin A OX-A Orexin receptors –
Orexins Orexin B OX-B Orexin receptors –
RFamides Kisspeptin KiSS GPR54 –
RFamides Neuropeptide FF NPFF NPFF1, NPFF2 –
RFamides Prolactin-releasing peptide PrRP PrRPR –
RFamides Pyroglutamylated RFamide peptide QRFP GPR103 –
Secretins Secretin Secretin receptor –
Secretins Motilin Motilin receptor –
Secretins Glucagon Glucagon receptor –
Secretins Glucagon-like peptide-1 GLP-1 Glucagon-like peptide 1 receptor –
Secretins Glucagon-like peptide-2 GLP-2 Glucagon-like peptide 2 receptor –
Secretins Vasoactive intestinal peptide VIP Vasoactive intestinal peptide receptors –
Secretins Growth hormone–releasing hormone GHRH Growth hormone–releasing hormone receptor –
Secretins Pituitary adenylate cyclase-activating peptide PACAP ADCYAP1R1 –
Somatostatins Somatostatin Somatostatin receptors –
Tachykinins Neurokinin A – –
Tachykinins Neurokinin B – –
Tachykinins Substance P – –
Tachykinins Neuropeptide K – –
Other Agouti-related peptide AgRP Melanocortin receptor –
Other N-Acetylaspartylglutamate NAAG Metabotropic glutamate receptor 3 (mGluR3) –
Other Cocaine- and amphetamine-regulated transcript CART Unknown Gi/Go-coupled receptor[16] –
Other Gonadotropin-releasing hormone GnRH GnRHR –
Other Thyrotropin-releasing hormone TRH TRHR –
Other Melanin-concentrating hormone MCH MCHR 1,2 –
Gasotransmitters
Category Name Abbreviation Metabotropic Ionotropic
Gaseous signaling molecule Nitric oxide NO Soluble guanylyl cyclase –
Gaseous signaling molecule Carbon monoxide CO – Heme bound to potassium channels
Gaseous signaling molecule Hydrogen sulfide H2S – –
Actions
Neurons form elaborate networks through which nerve impulses—action potentials—travel. Each neuron has as many as 15,000 connections with neighboring neurons.
Neurons do not touch each other (except in the case of an electrical synapse through a gap junction); instead, neurons interact at contact points called synapses: a junction within two nerve cells, consisting of a miniature gap within which impulses are carried by a neurotransmitter. A neuron transports its information by way of a nerve impulse called an action potential. When an action potential arrives at the synapse’s presynaptic terminal button, it may stimulate the release of neurotransmitters. These neurotransmitters are released into the synaptic cleft to bind onto the receptors of the postsynaptic membrane and influence another cell, either in an inhibitory or excitatory way. The next neuron may be connected to many more neurons, and if the total of excitatory influences minus inhibitory influences is great enough, it will also “fire”. That is to say, it will create a new action potential at its axon hillock, releasing neurotransmitters and passing on the information to yet another neighboring neuron.
Excitatory and inhibitory
A neurotransmitter can influence the function of a neuron through a remarkable number of mechanisms. In its direct actions in influencing a neuron’s electrical excitability, however, a neurotransmitter acts in only one of two ways: excitatory or inhibitory. A neurotransmitter influences trans-membrane ion flow either to increase (excitatory) or to decrease (inhibitory) the probability that the cell with which it comes in contact will produce an action potential. Thus, despite the wide variety of synapses, they all convey messages of only these two types, and they are labeled as such. Type I synapses are excitatory in their actions, whereas type II synapses are inhibitory. Each type has a different appearance and is located on different parts of the neurons under its influence.
Type I (excitatory) synapses are typically located on the shafts or the spines of dendrites, whereas type II (inhibitory) synapses are typically located on a cell body. In addition, Type I synapses have round synaptic vesicles, whereas the vesicles of type II synapses are flattened. The material on the presynaptic and post-synaptic membranes is denser in a Type I synapse than it is in a type II, and the type I synaptic cleft is wider. Finally, the active zone on a Type I synapse is larger than that on a Type II synapse.
The different locations of type I and type II synapses divide a neuron into two zones: an excitatory dendritic tree and an inhibitory cell body. From an inhibitory perspective, excitation comes in over the dendrites and spreads to the axon hillock to trigger an action potential. If the message is to be stopped, it is best stopped by applying inhibition on the cell body, close to the axon hillock where the action potential originates. Another way to conceptualize excitatory–inhibitory interaction is to picture excitation overcoming inhibition. If the cell body is normally in an inhibited state, the only way to generate an action potential at the axon hillock is to reduce the cell body’s inhibition. In this “open the gates” strategy, the excitatory message is like a racehorse ready to run down the track, but first the inhibitory starting gate must be removed.[17]
Examples of important neurotransmitter actions
As explained above, the only direct action of a neurotransmitter is to activate a receptor. Therefore, the effects of a neurotransmitter system depend on the connections of the neurons that use the transmitter, and the chemical properties of the receptors that the transmitter binds to.
Here are a few examples of important neurotransmitter actions:
Glutamate is used at the great majority of fast excitatory synapses in the brain and spinal cord. It is also used at most synapses that are “modifiable”, i.e. capable of increasing or decreasing in strength. Modifiable synapses are thought to be the main memory-storage elements in the brain. Excessive glutamate release can overstimulate the brain and lead to excitotoxicity causing cell death resulting in seizures or strokes.[18] Excitotoxicity has been implicated in certain chronic diseases including ischemic stroke, epilepsy, amyotrophic lateral sclerosis, Alzheimer’s disease, Huntington disease, and Parkinson’s disease.[19]
GABA is used at the great majority of fast inhibitory synapses in virtually every part of the brain. Many sedative/tranquilizing drugs act by enhancing the effects of GABA.[20] Correspondingly, glycine is the inhibitory transmitter in the spinal cord.
Acetylcholine was the first neurotransmitter discovered in the peripheral and central nervous systems. It activates skeletal muscles in the somatic nervous system and may either excite or inhibit internal organs in the autonomic system.[10] It is distinguished as the transmitter at the neuromuscular junction connecting motor nerves to muscles. The paralytic arrow-poison curare acts by blocking transmission at these synapses. Acetylcholine also operates in many regions of the brain, but using different types of receptors, including nicotinic and muscarinic receptors.[21]
Dopamine has a number of important functions in the brain; this includes regulation of motor behavior, pleasures related to motivation and also emotional arousal. It plays a critical role in the reward system; Parkinson’s disease has been linked to low levels of dopamine and schizophrenia has been linked to high levels of dopamine.[22]
Serotonin is a monoamine neurotransmitter. Most is produced by and found in the intestine (approximately 90%), and the remainder in central nervous system neurons. It functions to regulate appetite, sleep, memory and learning, temperature, mood, behaviour, muscle contraction, and function of the cardiovascular system and endocrine system. It is speculated to have a role in depression, as some depressed patients are seen to have lower concentrations of metabolites of serotonin in their cerebrospinal fluid and brain tissue.[23]
Norepinephrine which is synthesized in the central nervous system and sympathetic nerves, modulates the responses of the autonomic nervous system, the sleep patterns, focus and alertness.
It is synthesized from tyrosine
Epinephrine which is also synthesized from tyrosine is released in the adrenal glands and the brainstem.
It plays a role in sleep, with ones ability to become and stay alert, and the fight-or-flight response.
Histamine works with the central nervous system (CNS), specifically the hypothalamus (tuberomammillary nucleus) and CNS mast cells.
Brain neurotransmitter systems
Neurons expressing certain types of neurotransmitters sometimes form distinct systems, where activation of the system affects large volumes of the brain, called volume transmission. Major neurotransmitter systems include the noradrenaline (norepinephrine) system, the dopamine system, the serotonin system, and the cholinergic system, among others. Trace amines have a modulatory effect on neurotransmission in monoamine pathways (i.e., dopamine, norepinephrine, and serotonin pathways) throughout the brain via signaling through trace amine-associated receptor 1.[24][25] A brief comparison of these systems follows:
Neurotransmitter systems in the brain
System Pathway origin and projections Regulated cognitive processes and behaviors
Noradrenaline system
[26][27][28][29][30][31] Noradrenergic pathways:
Locus coeruleus (LC) projections
LC → Amygdala and Hippocampus
LC → Brain stem and Spinal cord
LC → Cerebellum
LC → Cerebral cortex
LC → Hypothalamus
LC → Tectum
LC → Thalamus
LC → Ventral tegmental area
Lateral tegmental field (LTF) projections
LTF → Brain stem and Spinal cord
LTF → Olfactory bulb
anxiety
arousal (wakefulness)
circadian rhythm
cognitive control and working memory (co-regulated by dopamine)
feeding and energy homeostasis
medullary control of respiration
negative emotional memory
nociception (perception of pain)
reward (minor role)
Dopamine system
[28][29][30][32][33][34] Dopaminergic pathways:
Ventral tegmental area (VTA) projections
VTA → Amygdala
VTA → Cingulate cortex
VTA → Hippocampus
VTA → Ventral striatum (Mesolimbic pathway)
VTA → Olfactory bulb
VTA → Prefrontal cortex (Mesocortical pathway)
Nigrostriatal pathway
Substantia nigra pars compacta → Dorsal striatum
Tuberoinfundibular pathway
Arcuate nucleus → Median eminence
Hypothalamospinal projection
Hypothalamus → Spinal cord
Incertohypothalamic pathway
Zona incerta → Hypothalamus
arousal (wakefulness)
aversion
cognitive control and working memory (co-regulated by norepinephrine)
emotion and mood
motivation (motivational salience)
motor function and control
positive reinforcement
reward (primary mediator)
sexual arousal, orgasm, and refractory period (via neuroendocrine regulation)
Histamine system
[29][30][35] Histaminergic pathways:
Tuberomammillary nucleus (TMN) projections
TMN → Cerebral cortex
TMN → Hippocampus
TMN → Neostriatum
TMN → Nucleus accumbens
TMN → Amygdala
TMN → Hypothalamus
arousal (wakefulness)
feeding and energy homeostasis
learning
memory
Serotonin system
[26][28][29][30][36][37][38] Serotonergic pathways:
Caudal nuclei (CN):
Raphe magnus, raphe pallidus, and raphe obscurus
Caudal projections
CN → Cerebral cortex
CN → Thalamus
CN → Caudate-putamen and nucleus accumbens
CN → Substantia nigra and ventral tegmental area
CN → Cerebellum
CN → Spinal cord
Rostral nuclei (RN):
Nucleus linearis, dorsal raphe, medial raphe, and raphe pontis
Rostral projections
RN → Amygdala
RN → Cingulate cortex
RN → Hippocampus
RN → Hypothalamus
RN → Neocortex
RN → Septum
RN → Thalamus
RN → Ventral tegmental area
arousal (wakefulness)
body temperature regulation
emotion and mood, potentially including aggression
feeding and energy homeostasis
reward (minor role)
sensory perception
Acetylcholine system
[26][28][29][30][39] Cholinergic pathways:
Forebrain cholinergic nuclei (FCN):
Nucleus basalis of Meynert, medial septal nucleus, and diagonal band
Forebrain nuclei projections
FCN → Hippocampus
FCN → Cerebral cortex
FCN → Limbic cortex and sensory cortex
Brainstem cholinergic nuclei (BCN):
Pedunculopontine nucleus, laterodorsal tegmentum, medial habenula, and
parabigeminal nucleus
Brainstem nuclei projections
BCN → Ventral tegmental area
BCN → Thalamus
arousal (wakefulness)
emotion and mood
learning
motor function
motivation (motivational salience)
short-term memory
reward (minor role)
Drug effects
Understanding the effects of drugs on neurotransmitters comprises a significant portion of research initiatives in the field of neuroscience. Most neuroscientists involved in this field of research believe that such efforts may further advance our understanding of the circuits responsible for various neurological diseases and disorders, as well as ways to effectively treat and someday possibly prevent or cure such illnesses.[40][medical citation needed]
Drugs can influence behavior by altering neurotransmitter activity. For instance, drugs can decrease the rate of synthesis of neurotransmitters by affecting the synthetic enzyme(s) for that neurotransmitter. When neurotransmitter syntheses are blocked, the amount of neurotransmitters available for release becomes substantially lower, resulting in a decrease in neurotransmitter activity. Some drugs block or stimulate the release of specific neurotransmitters. Alternatively, drugs can prevent neurotransmitter storage in synaptic vesicles by causing the synaptic vesicle membranes to leak. Drugs that prevent a neurotransmitter from binding to its receptor are called receptor antagonists. For example, drugs used to treat patients with schizophrenia such as haloperidol, chlorpromazine, and clozapine are antagonists at receptors in the brain for dopamine. Other drugs act by binding to a receptor and mimicking the normal neurotransmitter. Such drugs are called receptor agonists. An example of a receptor agonist is morphine, an opiate that mimics effects of the endogenous neurotransmitter β-endorphin to relieve pain. Other drugs interfere with the deactivation of a neurotransmitter after it has been released, thereby prolonging the action of a neurotransmitter. This can be accomplished by blocking re-uptake or inhibiting degradative enzymes. Lastly, drugs can also prevent an action potential from occurring, blocking neuronal activity throughout the central and peripheral nervous system. Drugs such as tetrodotoxin that block neural activity are typically lethal.
Drugs targeting the neurotransmitter of major systems affect the whole system, which can explain the complexity of action of some drugs. Cocaine, for example, blocks the re-uptake of dopamine back into the presynaptic neuron, leaving the neurotransmitter molecules in the synaptic gap for an extended period of time. Since the dopamine remains in the synapse longer, the neurotransmitter continues to bind to the receptors on the postsynaptic neuron, eliciting a pleasurable emotional response. Physical addiction to cocaine may result from prolonged exposure to excess dopamine in the synapses, which leads to the downregulation of some post-synaptic receptors. After the effects of the drug wear off, an individual can become depressed due to decreased probability of the neurotransmitter binding to a receptor. Fluoxetine is a selective serotonin re-uptake inhibitor (SSRI), which blocks re-uptake of serotonin by the presynaptic cell which increases the amount of serotonin present at the synapse and furthermore allows it to remain there longer, providing potential for the effect of naturally released serotonin.[41]
AMPT prevents the conversion of tyrosine to L-DOPA, the precursor to dopamine;
reserpine prevents dopamine storage within vesicles;
and deprenyl inhibits monoamine oxidase (MAO)-B and thus increases dopamine levels
.
Drug-Neurotransmitter Interactions[42]
Drug Interacts with: Receptor Interaction: Type Effects
Botulinum Toxin (Botox) Acetylcholine – Antagonist Blocks acetylcholine release in PNS
Prevents muscle contractions
Black Widow Spider Venom Acetylcholine – Agonist Promotes acetylcholine release in PNS
Stimulates muscle contractions
Neostigmine Acetylcholine – – Interferes with acetylcholinerase activity
Increases effects of ACh at receptors
Used to treat myasthenia gravis
Nicotine Acetylcholine Nicotinic (skeletal muscle) Agonist Increases ACh activity
Increases attention
Reinforcing effects
d-tubocurarine Acetylcholine Nicotinic (skeletal muscle) Antagonist Decreases activity at receptor site
Curare Acetylcholine Nicotinic (skeletal muscle) Antagonist Decreases ACh activity
Prevents muscle contractions
Muscarine Acetylcholine Muscarinic (heart and smooth muscle) Agonist Increases ACh activity
Toxic
Atropine Acetylcholine Muscarinic (heart and smooth muscle) Antagonist Blocks pupil constriction
Blocks saliva production
Scopolamine (Hyoscine) Acetylcholine Muscarinic (heart and smooth muscle) Antagonist Treats motion sickness and postoperative nausea and vomiting
.
AMPT
Dopamine/norepinephrine – –
Inactivates tyrosine hydroxylase and inhibits dopamine production
.
Reserpine Dopamine – – Prevents storage of dopamine and other monoamines in synaptic vesicles
Causes sedation and depression
Apomorphine Dopamine D2 Receptor (presynaptic autoreceptors/postsynaptic receptors) Antagonist (low dose)/Direct agonist (high dose) Low dose: blocks autoreceptors
High dose: stimulates postsynaptic receptors
Amphetamine Dopamine/norepinephrine – Indirect agonist Releases dopamine, noradrenaline, and serotonin
Blocks reuptake[24][25]
Methamphetamine Dopamine/norepinephrine – – Releases dopamine and noradrenaline
Blocks reuptake
Methylphenidate Dopamine – – Blocks reuptake
Enhances attention and impulse control in ADHD
Cocaine Dopamine – Indirect Agonist Blocks reuptake into presynapse
Blocks voltage-dependent sodium channels
Can be used as a topical anesthetic (eye drops)
Deprenyl Dopamine – Agonist Inhibits MAO-B
Prevents destruction of dopamine
Chlorpromazine Dopamine D2 Receptors Antagonist Blocks D2 receptors
Alleviates hallucinations
MPTP Dopamine – – Results in Parkinson like symptoms
PCPA Serotonin (5-HT) – Antagonist Disrupts serotonin synthesis by blocking the activity of tryptophan hydroxylase
Ondansetron Serotonin (5-HT) 5-HT3 receptors Antagonist Reduces side effects of chemotherapy and radiation
Reduces nausea and vomiting
Buspirone Serotonin (5-HT) 5-HT1A receptors Partial Agonist Treats symptoms of anxiety and depression
Fluoxetine Serotonin (5-HT) supports 5-HT reuptake SSRI Inhibits reuptake of serotonin
Treats depression, some anxiety disorders, and OCD[41] Common examples: Prozac and Sarafem
Fenfluramine Serotonin (5-HT) – – Causes release of serotonin
Inhibits reuptake of serotonin
Used as an appetite suppressant
Lysergic acid diethylamide Serotonin (5-HT) Post-synaptic 5-HT2A receptors Direct Agonist Produces visual perception distortions
Stimulates 5-HT2A receptors in forebrain
Methylenedioxymethamphetamine (MDMA) Serotonin (5-HT)/ norepinphrine – – Stimulates release of serotonin and norepinephrine and inhibits the reuptake
Causes excitatory and hallucinogenic effects
Strychnine Glycine – Antagonist Causes severe muscle spasms[43]
Diphenyldramine Histamine Crosses blood brain barrier to cause drowsiness
Tetrahydrocannabinol (THC) Endocannabinoids Cannabinoid (CB) receptors Agonist Produces analgesia and sedation
Increases appetite
Cognitive effects
Rimonabant Endocannabinoids Cannabinoid (CB) receptors Antagonist Suppresses appetite
Used in smoking cessation
MAFP Endocannabinoids – – Inhibits FAAH
Used in research to increase cannabinoid system activity
AM1172 Endocannabinoids – – Blocks cannabinoid reuptake
Used in research to increase cannabinoid system activity
Anandamide (endogenous) – Cannabinoid (CB) receptors; 5-HT3 receptors – Reduce nausea and vomiting
Caffeine Adenosine Adenosine receptors Antagonist Blocks adenosine receptors
Increases wakefulness
PCP Glutamate NMDA receptor Indirect Antagonist Blocks PCP binding site
Prevents calcium ions from entering neurons
Impairs learning
AP5 Glutamate NMDA receptor Antagonist Blocks glutamate binding site on NMDA receptor
Impairs synaptic plasticity and certain forms of learning
NMDA Glutamate NMDA receptor Agonist Used in research to study NMDA receptor
Ionotropic receptor
AMPA Glutamate AMPA receptor Agonist Used in research to study AMPA receptor
Ionotropic receptor
Ketamine Glutamate Kainate receptor Antagonist Used in research to study Kainate receptor
Induces trance-like state, helps with pain relief and sedation
Allyglycine GABA – – Inhibits GABA synthesis
Causes seizures
Muscimol GABA GABA receptor Agonist Causes sedation
Bicuculine GABA GABA receptor Antagonist Causes Seizures
Benzodiazepines GABA GABAA receptor Indirect agonists Anxiolytic, sedation, memory impairment, muscle relaxation
Barbiturates GABA GABAA receptor Indirect agonists Sedation, memory impairment, muscle relaxation
Alcohol GABA GABA receptor Indirect agonist Sedation, memory impairment, muscle relaxation
Picrotoxin GABA GABAA receptor Indirect antagonist High doses cause seizures
Tiagabine GABA – Antagonist GABA transporter antagonist
Increase availability of GABA
Reduces the likelihood of seizures
Moclobemide Norepinephrine – Agonist Blocks MAO-A to treat depression
Idazoxan Norepinephrine alpha-2 adrenergic autoreceptors Agonist Blocks alpha-2 autoreceptors
Used to study norepinephrine system
Fusaric acid Norepinephrine – – Inhibits activity of dopamine beta-hydroxylase which blocks the production of norepinephrine
Used to study norepinephrine system without affecting dopamine system
Opiates (Opium, morphine, heroin,and oxycodone) Opioids Opioid receptor[44] Agonists Analgesia, sedation, and reinforcing effects
Naloxone Opioids – Antagonist Reverses opiate intoxication or overdose symptoms (i.e. problems with breathing)
This section needs expansion with: coverage of full agonists and their distinction from partial agonist and inverse agonist..
You can help by . (August 2015)
An agonist is a chemical capable of binding to a receptor, such as a neurotransmitter receptor, and initiating the same reaction typically produced by the binding of the endogenous substance.[45] An agonist of a neurotransmitter will thus initiate the same receptor response as the transmitter. In neurons, an agonist drug may activate neurotransmitter receptors either directly or indirectly.
Direct-binding agonists can be further characterized as full agonists, partial agonists, inverse agonists.[citation needed]
Direct agonists act similar to a neurotransmitter by binding directly to its associated receptor site(s), which may be located on the presynaptic neuron or postsynaptic neuron, or both.[46] Typically, neurotransmitter receptors are located on the postsynaptic neuron, while neurotransmitter autoreceptors are located on the presynaptic neuron, as is the case for monoamine neurotransmitters;[24] in some cases, a neurotransmitter utilizes retrograde neurotransmission, a type of feedback signaling in neurons where the neurotransmitter is released postsynaptically and binds to target receptors located on the presynaptic neuron.[47][note 1] Nicotine, a compound found in tobacco, is a direct agonist of most nicotinic acetylcholine receptors, mainly located in cholinergic neurons.[44] Opiates, such as morphine, heroin, hydrocodone, oxycodone, codeine, and methadone, are μ-opioid receptor agonists; this action mediates their euphoriant and pain relieving properties.[44]
Indirect agonists increase the binding of neurotransmitters at their target receptors by stimulating the release or preventing the reuptake of neurotransmitters.[46] Some indirect agonists trigger neurotransmitter release and prevent neurotransmitter reuptake. Amphetamine, for example, is an indirect agonist of postsynaptic dopamine, norepinephrine, and serotonin receptors in each their respective neurons;[24][25] it produces both neurotransmitter release into the presynaptic neuron and subsequently the synaptic cleft and prevents their reuptake from the synaptic cleft by activating TAAR1, a presynaptic G protein-coupled receptor, and binding to a site on VMAT2, a type of monoamine transporter located on synaptic vesicles within monoamine neurons.[24][25]
An antagonist is a chemical that acts within the body to reduce the physiological activity of another chemical substance (as an opiate); especially one that opposes the action on the nervous system of a drug or a substance occurring naturally in the body by combining with and blocking its nervous receptor.[48]
There are two main types of antagonist: direct-acting Antagonist and indirect-acting Antagonists:
Direct-acting antagonist- which takes up space present on receptors which are otherwise taken up by neurotransmitters themselves. This results in neurotransmitters being blocked from binding to the receptors. The most common is called Atropine.
Indirect-acting antagonist- drugs that inhibit the release/production of neurotransmitters (e.g., Reserpine).
Drug antagonists
An antagonist drug is one that attaches (or binds) to a site called a receptor without activating that receptor to produce a biological response. It is therefore said to have no intrinsic activity. An antagonist may also be called a receptor “blocker” because they block the effect of an agonist at the site. The pharmacological effects of an antagonist therefore result in preventing the corresponding receptor site’s agonists (e.g., drugs, hormones, neurotransmitters) from binding to and activating it. Antagonists may be “competitive” or “irreversible”.
A competitive antagonist competes with an agonist for binding to the receptor. As the concentration of antagonist increases, the binding of the agonist is progressively inhibited, resulting in a decrease in the physiological response. High concentration of an antagonist can completely inhibit the response. This inhibition can be reversed, however, by an increase of the concentration of the agonist, since the agonist and antagonist compete for binding to the receptor. Competitive antagonists, therefore, can be characterized as shifting the dose–response relationship for the agonist to the right. In the presence of a competitive antagonist, it takes an increased concentration of the agonist to produce the same response observed in the absence of the antagonist.
An irreversible antagonist binds so strongly to the receptor as to render the receptor unavailable for binding to the agonist. Irreversible antagonists may even form covalent chemical bonds with the receptor. In either case, if the concentration of the irreversible antagonist is high enough, the number of unbound receptors remaining for agonist binding may be so low that even high concentrations of the agonist do not produce the maximum biological response.[49]
While intake of neurotransmitter precursors does increase neurotransmitter synthesis, evidence is mixed as to whether neurotransmitter release and postsynaptic receptor firing is increased. Even with increased neurotransmitter release, it is unclear whether this will result in a long-term increase in neurotransmitter signal strength, since the nervous system can adapt to changes such as increased neurotransmitter synthesis and may therefore maintain constant firing.[53][unreliable medical source?] Some neurotransmitters may have a role in depression and there is some evidence to suggest that intake of precursors of these neurotransmitters may be useful in the treatment of mild and moderate depression.[53][unreliable medical source?][54]
Catecholamine and trace amine precursors
L-DOPA, a precursor of dopamine that crosses the blood–brain barrier, is used in the treatment of Parkinson’s disease. For depressed patients where low activity of the neurotransmitter norepinephrine is implicated, there is only little evidence for benefit of neurotransmitter precursor administration.
L-phenylalanine and L-tyrosine are both precursors for dopamine, norepinephrine, and epinephrine.
These conversions require vitamin B6, vitamin C, and S-adenosylmethionine.
A few studies suggest potential antidepressant effects of L-phenylalanine and L-tyrosine, but there is much room for further research in this area.
[unreliable medical source?]
.
Serotonin precursors
Administration of L-tryptophan, a precursor for serotonin, is seen to double the production of serotonin in the brain.
It is significantly more effective than a placebo in the treatment of mild and moderate depression.[53][unreliable medical source?] This conversion requires vitamin C.[23] 5-hydroxytryptophan (5-HTP), also a precursor for serotonin, is more effective than a placebo.[53][unreliable medical source?]
Diseases and disorders
Diseases and disorders may also affect specific neurotransmitter systems. The following are disorders involved in either an increase, decrease, or imbalance of certain neurotransmitters.
Dopamine:
For example, problems in producing dopamine (mainly in the substantia nigra) can result in Parkinson’s disease, a disorder that affects a person’s ability to move as they want to, resulting in stiffness, tremors or shaking, and other symptoms. Some studies suggest that having too little or too much dopamine or problems using dopamine in the thinking and feeling regions of the brain may play a role in disorders like schizophrenia or attention deficit hyperactivity disorder (ADHD). Dopamine is also involved in addiction and drug use, as most recreational drugs cause an influx of dopamine in the brain (especially opioid and methamphetamines) that produces a pleasurable feeling, which is why users constantly crave drugs.
Serotonin:
Similarly, after some research suggested that drugs that block the recycling, or reuptake, of serotonin seemed to help some people diagnosed with depression, it was theorized that people with depression might have lower-than-normal serotonin levels. Though widely popularized, this theory was not borne out in subsequent research.[55] Therefore, selective serotonin reuptake inhibitors (SSRIs) are used to increase the amounts of serotonin in synapses.
Glutamate:
Furthermore, problems with producing or using glutamate have been suggestively and tentatively linked to many mental disorders, including autism, obsessive compulsive disorder (OCD), schizophrenia, and depression.[56] Having too much glutamate has been linked to neurological diseases such as Parkinson’s disease, multiple sclerosis, Alzheimer’s disease, stroke, and ALS (amyotrophic lateral sclerosis).[57]
CAPON Binds Nitric Oxide Synthase, Regulating NMDA Receptor–Mediated Glutamate Neurotransmission
Neurotransmitter imbalance
Generally, there are no scientifically established “norms” for appropriate levels or “balances” of different neurotransmitters. It is in most cases pragmatically impossible to even measure levels of neurotransmitters in a brain or body at any distinct moments in time. Neurotransmitters regulate each other’s release, and weak consistent imbalances in this mutual regulation were linked to temperament in healthy people .[58][59][60][61][62] Strong imbalances or disruptions to neurotransmitter systems have been associated with many diseases and mental disorders. These include Parkinson’s, depression, insomnia, Attention Deficit Hyperactivity Disorder (ADHD), anxiety, memory loss, dramatic changes in weight and addictions. Chronic physical or emotional stress can be a contributor to neurotransmitter system changes. Genetics also plays a role in neurotransmitter activities. Apart from recreational use, medications that directly and indirectly interact one or more transmitter or its receptor are commonly prescribed for psychiatric and psychological issues. Notably, drugs interacting with serotonin and norepinephrine are prescribed to patients with problems such as depression and anxiety—though the notion that there is much solid medical evidence to support such interventions has been widely criticized.[63] Studies shown that dopamine imbalance has an influence on multiple sclerosis and other neurological disorders
.
Elimination of neurotransmitters
A neurotransmitter must be broken down once it reaches the post-synaptic cell to prevent further excitatory or inhibitory signal transduction.
This allows new signals to be produced from the adjacent nerve cells. When the neurotransmitter has been secreted into the synaptic cleft, it binds to specific receptors on the postsynaptic cell, thereby generating a postsynaptic electrical signal. The transmitter must then be removed rapidly to enable the postsynaptic cell to engage in another cycle of neurotransmitter release, binding, and signal generation. Neurotransmitters are terminated in three different ways:
Diffusion –
the neurotransmitter detaches from receptor, drifting out of the synaptic cleft, here it becomes absorbed by glial cells.
Enzyme degradation –
special chemicals called enzymes break it down.
Usually, astrocytes absorb the excess neurotransmitters and pass them on to enzymes or pump them directly into the presynaptic neuron.
Reuptake –
re-absorption of a neurotransmitter into the neuron.
Transporters, or membrane transport proteins, pump neurotransmitters from the synaptic cleft back into axon terminals (the presynaptic neuron) where they are stored.
For example, choline is taken up and recycled by the pre-synaptic neuron to synthesize more ACh.
Other neurotransmitters such as dopamine are able to diffuse away from their targeted synaptic junctions and are eliminated from the body via the kidneys, or destroyed in the liver.
.
(each ‘neuro-transmitter’ has very specific ‘degradation pathways’ at ‘regulatory points’, which may be targeted by the body’s ‘regulatory system’ or by ‘recreational drugs’)
.
.
.
.
.
.
.
.
.
.
.